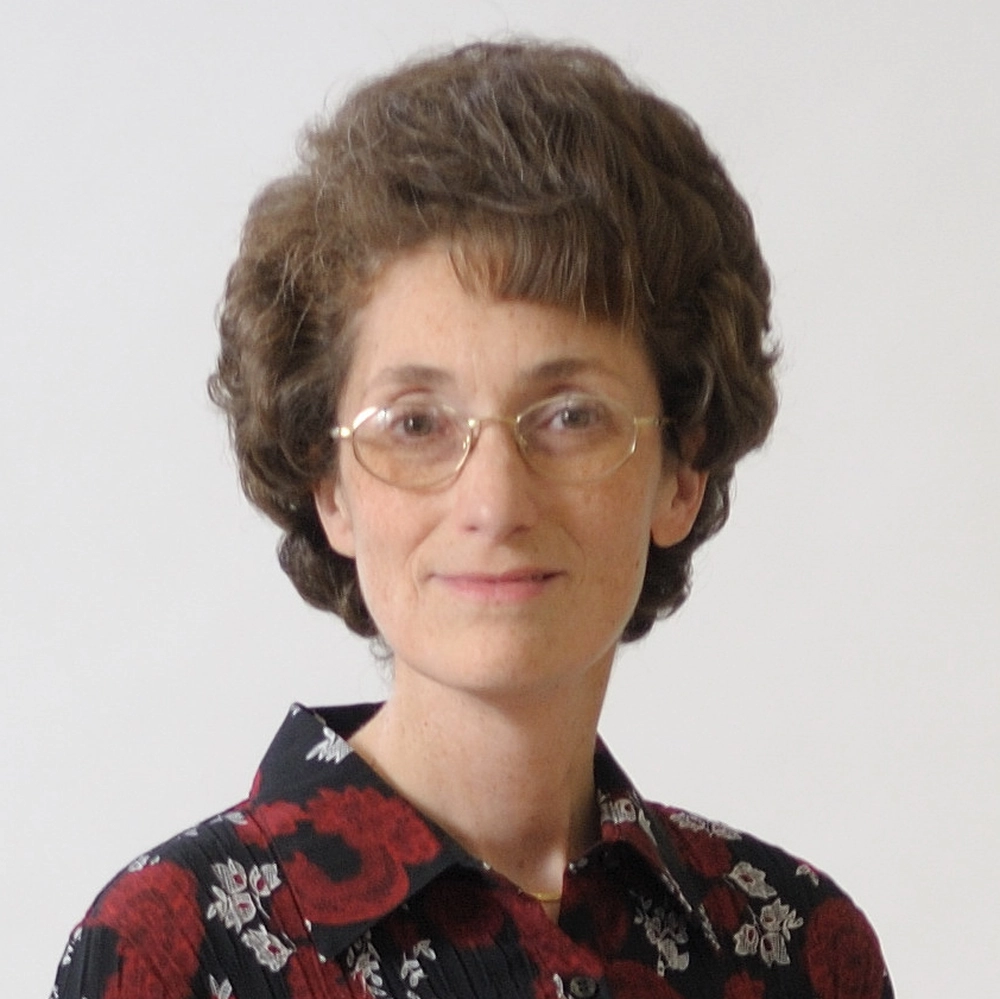
By John H. Burke
For over a decade, scientists have debated the role of quantum mechanical coherences in photosynthesis. Now, using high-level quantum dynamics simulations, graduate student Sohang Kundu and Nancy Makri, Edward William and Jane Marr Gutgsell Chair and Professor of Chemistry, have provided strong insight into nature’s ability to harness quantum mechanics to process light energy into chemical energy.
Makri and Kundu have produced the first fully quantum mechanical simulation of the energy transfer process in photosynthetic light-harvesting complexes that uses an accurate description of the pigment molecular vibrations, which were obtained from experimental spectra. Their work, which was published in The Journal of Physical Chemistry Letters, tries to answer how long electronic coherences survive in photosynthetic complexes.
Quantum coherences are delicate phenomena, and their role in photosynthesis has been hotly debated in the scientific world.
Some ultrafast 2D spectroscopy experiments of photosynthetic light-harvesting complexes have provided evidence for quantum coherences, or superpositions of quantum states, in the form of oscillatory features in the time-dependent signals. Based on that evidence, models of wavelike energy transfer have been proposed.
Light-harvesting complexes are protein complexes with carefully arranged arrays of pigment molecules which absorb light and transfer the excitation energy between each other and ultimately to a reaction center. In the wavelike energy transfer model, the excitation energy propagates between pigment molecules like a wave, instead of discretely hopping between the pigment molecules. This is an example of an electronic coherence, or a quantum superposition of electronic states, the existence of which in a biological system would be quite remarkable, according to the researchers.
Electronic coherences are extremely delicate, and because of decoherence in the environment, typically persist no longer than 100 femtoseconds (fs, 10-15 s). Furthermore, the protein environment surrounding the pigment molecules in photosynthetic light-harvesting complexes features many atoms at ambient temperature, which are able to move between many thermally accessible conformations. This makes the surrounding protein environment a very efficient thermal bath and a strong source of decoherence. For those reasons, one would not expect electronic coherences to last long in photosynthetic light-harvesting complexes.
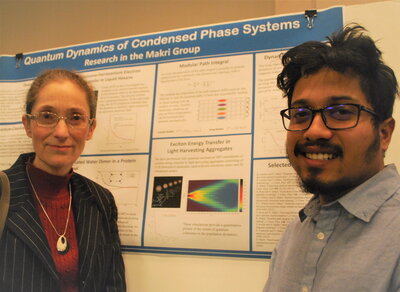
Yet, some ultrafast 2D spectroscopy experiments provided evidence of electronic coherences persisting for 1.5 picoseconds (ps, 10-12 s). These surprising observations led scientists to question whether nature evolved to protect these delicate quantum states from dephasing from the environment, for example, by tailoring the protein-pigment interactions.
Long-lived electronic coherences in photosynthetic light-harvesting complexes have remained controversial, sparking a huge thrust of research in the area of quantum biology. This latest research of Kundu and Makri brings new insight to this phenomenon through highly-accurate quantum dynamics simulations that incorporate molecular vibrations at room temperature.
Kundu and Makri’s simulation addresses how long electronic coherence can survive in the B850 ring of LH2, a light-harvesting complex of purple photosynthetic bacteria. The B850 ring is a ring of 18 bacteriochlorophyll pigment molecules, which are carefully arranged to absorb light and transfer the excitation energy between molecules.
The team simulated the B850 ring in the absence of the protein surroundings, eliminating a large source of decoherence and allowing them to examine the decoherence caused by the vibrations of the pigment molecules themselves.
“The question we were trying to answer is how dissipative the dynamics would be just in the presence of vibrations themselves, and if vibrations can add to coherences or if they only remove coherences,” said Kundu.
What makes their study stand out is the number of vibrations that they treated. For each of the 18 bacteriochlorophyll pigment molecules in the ring, they treated 50 vibrational modes. This represents a much more accurate picture than other past studies, which in some cases only treat a single vibrational mode per molecule.
They were able to treat so many vibrations by using the modular path integral (MPI) method. The MPI method, based on Richard Feynman’s path integral formalism, allows for the simulation of complex systems at lower computational cost than wavefunction methods. “The problem with wavefunction methods is that the computational cost scales exponentially with the number of vibrational states considered,” Kundu explains. “The MPI method, on the other hand, allows us to treat as many vibrations as we want, at whatever temperature we want, without adding any significant cost to our calculations.”
In addition to treating 50 vibrations, their model represents a highly accurate one in that they don’t make any approximation about the electronic-vibrational coupling. Each molecule is modeled as having an electronic ground and excited state, the energy of which they obtained from high-level electronic structure calculations published in the literature. Each of the 50 vibrational modes is coupled by some amount to this electronic transition, and they used couplings from published fluorescence line narrowing experiments to avoid making approximations. By using experimental values for the coupling, they create a highly accurate model of the pigment ring.
The simulations, which were run for 100 fs on a 3 fs grid, were performed on the School of Chemical Sciences computer cluster and the Makri Group’s dedicated computer cluster.
They simulate the energy transfer as follows: one pigment in the ring starts off in an electronic excited state at the geometry of the ground state, representing a molecule that has just absorbed a photon of light. Then, the clock starts, and the energy begins to spread out along the ring like a wave (this is the electronic coherence). In the absence of the vibrations, this wave continues to propagate indefinitely and interferes with itself.
In the presence of the vibrations, however, the wavelike oscillations of excited state population are heavily damped out. By the end of the simulation at 100 fs, hardly any wavelike oscillations are observed, suggesting efficient decoherence by the molecular vibrations.
“Even in the absence of the protein, these coherences do not last longer than 100 fs,” Kundu said.
This serves as an upper bound for the electronic coherence in the real system, because consideration of the protein environment would further contribute to the decoherence.
Their results show that some electronic coherences are observed at short times, but the molecular vibrations are enough to cause decoherence within 100 fs. However, they observed persistent oscillatory patterns in shorter bacteriochlorophyll aggregates that they simulated. The team speculates about what functional role this amount of decoherence has in photosynthesis.
“What we’re finding is that in the isolated ring without the protein environment, the energy transfer dynamics is borderline between being overdamped and underdamped,” Makri said. “We think this helps the spreading of the excitation energy around the ring.”
If the dynamics are underdamped and the coherence is long-lived, then the excited-state population of the pigments will oscillate with time. If the dynamics are overdamped, then the dynamics will likely be slower.
They also wonder how this level of decoherence affects transfer of excitation energy from one ring of pigment molecules to another. The LH2 complex features two rings of pigment molecules: the B800 ring with 9 bacteriochlorophyll molecules, and the B850 ring with 18 bacteriochlorophyll molecules. When the B800 rings absorbs light, the excitation energy is eventually transferred to the B850 ring.
"The next question we are pursuing is whether this level of decoherence helps the transfer of energy from ring-to-ring," Makri said.
Although considering both coupled rings of bacteriochlorophyll drastically increases the size of the system, the path integral methods the group has developed makes this calculation feasible.
Other future work includes modeling 2D electronic spectra.
"As of now, we have only studied the population dynamics and coherences as a function of time. The next step would be to simulate two-dimensional spectroscopy experiments," Kundu said.
Noting that 2D spectra can be very difficult to interpret, Makri wants to answer the question “can we take a 2D spectrum and process the information and get the population dynamics from it.” Doing so would represent a huge milestone in uncovering the role of quantum coherences in photosynthesis.
Makri is also a professor of physics and a member of the Center for Synthesizing Quantum Coherence, a Phase I Center for Chemical Innovation funded by the National Science Foundation for the purpose of unmasking the coherent quantum mechanical flow of electronic excited states and of charges, along with their coherent spin-spin interactions, in precisely tailored nanostructures and molecules.
Kundu, S.; Makri, N. Real-Time Path Integral Simulation of Exciton-Vibration Dynamics in Light-Harvesting Bacteriochlorophyll Aggregates. J. Phys. Chem. Lett. 2020, 11, 20, 8783–8789 https://doi.org/10.1021/acs.jpclett.0c02760